Ambient carbon dioxide concentration correlates with SARS-CoV-2 aerostability and infection risk
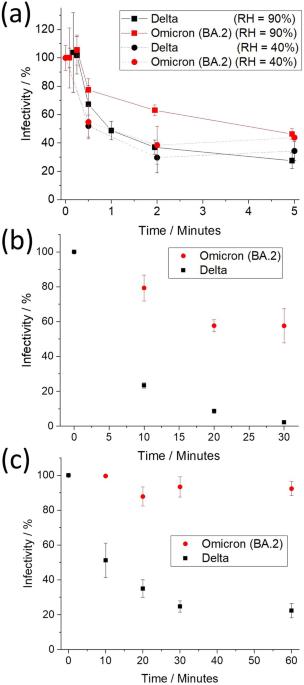
The BA.2 omicron VOC is more aerostable than the delta VOC
Previously, we reported that the aerostability of the VOCs of SARS-CoV-2 (wild type, Alpha, Beta, and Delta) correlated with the variant’s stability in an alkaline growth medium over short-time periods (under five minutes)15. Specifically, it was reported that as the virus has evolved from wild type through to Delta VOC, it has become both more sensitive to high pH and less aerostable. The relationship between pH and the aerostability of the (Omicron) BA.2 VOC is compared to the Delta VOC to further explore this comparison (Fig. 1). Firstly, the aerostabilities of the Delta and BA.2 VOCs within aerosol droplets are reported at moderate/low (40%) and high RH (90%) values in Fig. 1a. At 40% RH, the Delta and BA.2 VOCs exhibit similar decay profiles. This is consistent with our previous studies where all VOCs exhibited a similar decay profile when the RH is below the droplet efflorescence threshold, highlighted by the near-instantaneous loss of ~50% of viral infectivity associated with the efflorescence event. At 90% RH, the overall rate of decay of the BA.2 VOC is much slower than the Delta VOC. At 5 min, relative to the Delta VOC, the total viable aerosolized viral load of the BA.2 VOC is 1.7 times higher. Excluding data points in the transient decay (p = 3 × 10−4, with 19.8 effective degrees of freedom via Satterthwaite approximation) at 90% RH. This effect persists at the 5 min mark, with a difference of 19 ± 8 % (p = 0.03, with 18.9 degrees of freedom). At 90% RH, the general structures of the decay profiles for both VOCs are consistent with previous VOCs, with an initial lag period of ~15 s, followed by a rapid loss to ~2 min, and a more gradual subsequent decay.
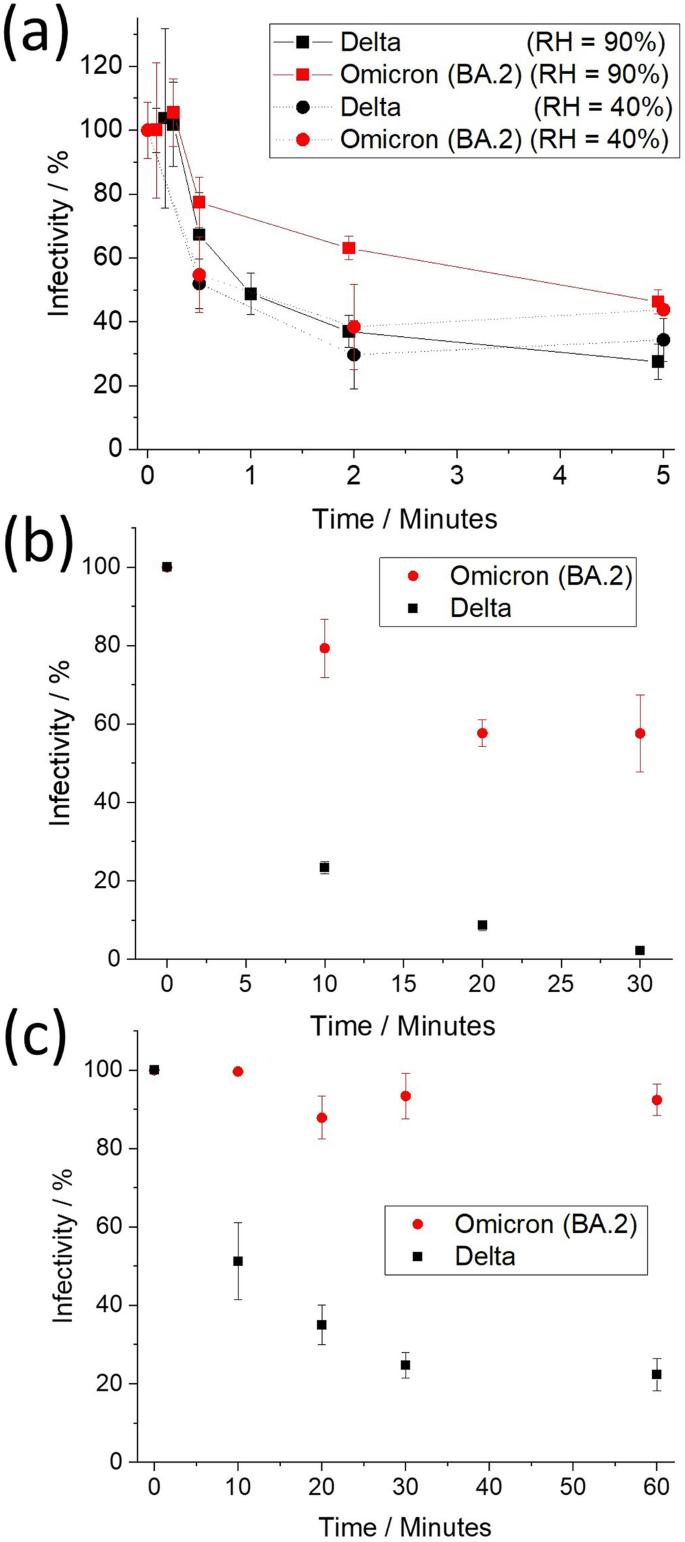
a Infectivity of the Delta and Omicron BA.2 VOCs that have been levitated at RHs of 40% and 90%. Data for 90% at times over 100 s are offset by 5 s to facilitate reader interpretation. b, c Infectivity of BA.2 and Delta VOCs, respectively, in DMEM 2% FBS bulk solution with pH maintained at 11 and measured by b cytopathy and c immunostaining. Values are means ± SE. Both b and c fit a first-order decay; from a t-test to compare slopes of regression lines (two-sided), the linear fits of ln(infectivity) vs. time for the Omicron and Delta VOCs are consistent with decay rates that are significant in their difference p = 0.0002 (n = 24 (independent samples)) for (b), p = 0.027 (n = 30 (independent samples)) for (c). Source data are provided as a Source Data file.
Under high alkaline conditions in the bulk phase, the BA.2 VOC is found to be more resistant to high pH conditions than the Delta VOC assessed via two different measurements of infectivity (Fig. 1b, c). This is consistent with the hypothesis that the differences between aerostability of the Delta and BA.2 VOCs are likely a consequence of their relative stability in a highly alkaline solution. BA.2 is the first VOC of SARS-CoV-2 that we have demonstrated to have an increase in stability at high pH when compared to a prior VOC. The microbiological mechanisms underlying these differences in pH sensitivity remain unclear and are in need of further research.
Collectively, the data shown in Fig. 1 support the hypothesis that high pH achieved in aerosol, with an initial composition that has high abundance of bicarbonate (such as saliva27 and growth medium), is a major factor in driving loss of viral infectivity in the aerosol phase15. The implication of this proposed mechanism is that any gaseous species (e.g., CO2) that can affect aerosol pH is likely to impact viral infectivity.
SARS-CoV-2 aerostability correlates with the ambient concentration of gas-phase carbon dioxide
In poorly ventilated, occupied, indoor spaces, ambient [CO2(g)] commonly reaches concentrations exceeding 2000 ppm28 and can reach levels upwards of >5000 ppm in more crowded environments29. The impact of elevated CO2(g) levels on the aerostability of SARS-CoV-2 is explored (Fig. 2). Both the sensitivity and the throughput of the CELEBS technique are dependent on the initial viral load of the individual droplets. The maximum titer of the BA.2 VOC that can be grown in the cell culture is approximately an order of magnitude less than that of the Delta VOC. Thus, in order to explore the effect that [CO2(g)] has on viral aerostability across a broad range of conditions and over long-time periods, the majority of the testing was conducted with the Delta VOC as it afforded a much higher measurement throughput.
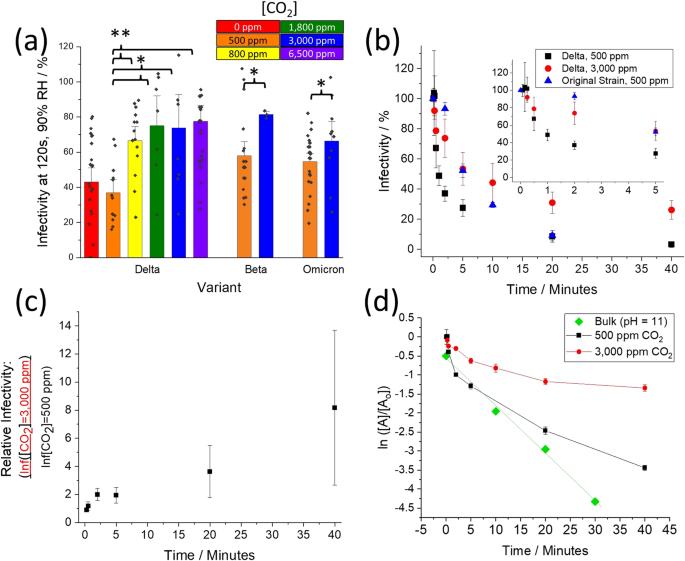
a Infectivity of the Delta, Beta, and Omicron BA.2 VOCs as a function of ambient concentrations of CO2(g) at 90% RH, 120 s. Statistical significance was assessed using a one-sided, two-sample equal variance, t-test (*p ≤ 0.05, **p ≤ 0.005, n = 146 (independent samples)). Specifically, the significance (p-value) between 500 ppm and 800 ppm, 1800 ppm, 3000 ppm, and 6500 ppm was 0.003, 0.025, 0.032, and 0.001, respectively. b The effect that an elevated concentration of CO2 has on the decay profile of the Delta VOC and original strain of SARS-CoV-2 at 90% RH. Inset is simply a zoom-in of the first 5 min of the x-axis. Elevating the [CO2(g)] results in a significant difference in overall decay assessed using a one-sided, two-sample equal variance, t-test (n = 188 (independent samples)) of the Delta VOC from 2 min onward, where the significance (p-value) was 0.007, 0.027, 0.020 and 0.005 for 2, 5, 10 and 40 min, respectively. c Relative infectivity of aerosolized Delta VOC exposed to increased [CO2(g)] as a function of time. The ratios were estimated using the raw data in Fig. 2b at time points where the infectivity was measured for both [CO2(g)]. Note that the error bars increase with time results from the infectivity of the “Delta, 500 ppm” data set approaching zero which causes the relative standard deviation to increase. d Infectivity of the Delta VOC (ln([A]/[Ao])) as a function of time in aerosol and in MEM at pH = 11 (bulk data offset by −0.5). Least square fit through the Bulk data (R2 = 0.995). Values are means ± SE. Source data are provided as a Source Data file.
When compared to a typical atmospheric [CO2(g)] (~500 ppm), increasing the [CO2(g)] to just 800 ppm results in a significant increase in viral aerostability after 2 min (Delta VOC, Fig. 2a). No significant difference in infectivity is observed between 800 ppm and 6500 ppm. It is notable that, according to the UK Scientific Advisory Group for Emergencies (SAGE), 800 ppm [CO2(g)] has been identified as the level below which a room is determined to be well-ventilated. When ambient airflow into the CELEBS is substituted with synthetic air (0 ppm [CO2(g)]), no change in virus aerostability is observed.
Increasing the [CO2(g)] results in a significant increase in the aerostability of both the Beta and Omicron BA.2 VOCs at 120 s. This suggests that the viral aerostability is dependent on CO2(g) concentration for all SARS-CoV-2 variants. The increase in infectivity of the Omicron variant due to the elevated [CO2] whilst similar, is slightly lower (+11.7%) than the Beta (+23.4%) and Delta (+36.8%) VOCs. This is likely a product of the differing pH sensitivities for different variants, with the more pH-sensitive variants more sensitive to changes in [CO2]. It is unlikely a similar increase across variants will occur at all time periods for all variants.
The rate of viral infectivity loss correlates with aerosol alkalinity. Elevated [CO2(g)] limits the amount of bicarbonate leaving the droplet (Eq. 1) and, thus, limits the maximum pH that the droplet will reach. A significant improvement in aerostability of SARS-CoV-2 resulting from elevated [CO2(g)] would be expected to increase over time as the droplet will spend less time at the elevated pH30. The effect of droplet exposure to elevated [CO2(g)] over prolonged time periods on the infectious viral load is reported in Fig. 2b and it can be seen that elevated [CO2(g)] had a considerable effect on the overall decay profile. Consider first the characteristics of the decay profile of the wild type SARS-CoV-2 in the aerosol phase above the efflorescence point14. From droplet generation until ~2 min, no loss of infectivity is observed. After 2 min there is a rapid loss of infectivity over a moderate time period (minutes), followed by a slower decay (tens of minutes). The profile for the Delta VOC is similar but with the initial lag period shortened to ~15 s. In this case, when the [CO2(g)] is elevated, the period of rapid decay is absent or greatly abbreviated and the decay profile transitions directly from lag to a slow decay. As a result, the Delta VOC in elevated [CO2(g)] is as aerostable as the wild type at 500 ppm CO2 after 5 min, resulting in a larger fraction remaining infectious after 20 min (p = 0.04). Indeed, elevated [CO2(g)] has a dramatic effect on the remaining relative infectivity of SARS-CoV-2 over time (Fig. 2c). A hypothesis test regression of slope for the data in Fig. 2c found a p = 0.00038, consistent with a lower rate of viral decay at elevated [CO2(g)]. After 40 min, approximately an order of magnitude more viral infectious particles remain viable in the aerosol phase at elevated [CO2(g)] when compared to the loss expected under ambient (well-ventilated) conditions. This increase in the relative abundance of infectious particles is likely to result in increased risk of transmission of the infection.
Viral aerostability is often reported as having a half-life31 with the decay assumed to follow first-order (exponential) reaction kinetics. This assumption presupposes that the mechanisms involved in infectivity loss do not change over time, even though the chemical composition and physical conditions inside an aerosol droplet vary over time. The appropriateness of making such assumptions is explored in Fig. 2d. In the bulk phase, the pH-driven decay follows first-order kinetics. This is consistent with high [OH−] driving the loss of viral infectivity, with this concentration remaining constant over time. However, the decay dynamics are markedly different in the aerosol phase with the rate of loss slowing over time, and slowing more so at higher [CO2(g)]. This is consistent with the hypothesis that the aerosol achieves high pH before being buffered towards a neutral pH by trace acidic vapor over longer time periods (condensable carbonic acid in this case), regardless of RH. However, the decay rate is never found to increase over the entire time period studied, suggesting that the pH of the aerosol does not pass through neutral to become acidic during the time period when more than 95% of the viral infectivity is lost. Again, this is consistent with the condensation of a weak acid, carbonic acid in this case. However, a half-life of ~80 min could be estimated from the data falling between 20 and 40 min at [CO2(g)] of 3000 ppm if one were to assume a first-order decay. This aligns with the half-lives reported using other measurement approaches in which the [CO2(g)] is neither measured nor controlled31.
Collectively, the data shown in Fig. 2 show that the interplay between aerosol alkalinity and CO2 has a profound effect on the overall aerostability of SARS-CoV-2. Any increase in [CO2(g)] results in an increase in aerostability.
Depending on variant pH sensitivity, ambient [CO2] and solute composition can affect viral aerostability more than relative humidity
During the COVID-19 pandemic, many infections were traced to super-spreader events32, suggesting that transmission of the virus over longer distances was possible under some (as yet uncertain) conditions1,33. Conversely, the apparent effectiveness of mitigation strategies such as social distancing regulations7, use of face shields/masks34, and installation of plexiglass shields35 suggests that SARS-CoV-2 was also commonly transmitted over short distances. Thus, it is important to understand how environmental factors affect the aerostability of SARS-CoV-2 over time periods as short as 15 s.
Fixing the time in the aerosol phase at 15 s, the BA.2 VOC is found to be more aerostable than the Delta VOC across a broad range of RH (Fig. 3a). Effectively, during the first 15 s post-aerosol generation there is no loss of infectivity of the BA.2 VOC when the RH is above the efflorescence point of the particle (RH~50%). Below that, the characteristic rapid loss of approximately half of the viral infectivity is observed, a consequence of the efflorescence event. This is consistent with the loss observed for the other SARS-CoV-2 VOCs we have studied14,15,36 as well as for mouse hepatitis virus (MHV), another coronavirus37. At an RH between ~50% and 80%, the Delta VOC is less aerostable than the other VOCs studied, rapidly losing over half of its infectivity within 15 s of aerosolization. Collectively, the data in Fig. 3a show that the initial decay of the Delta VOC is largely RH independent, while the initial decay of the BA.2 VOC is highly RH-dependent.
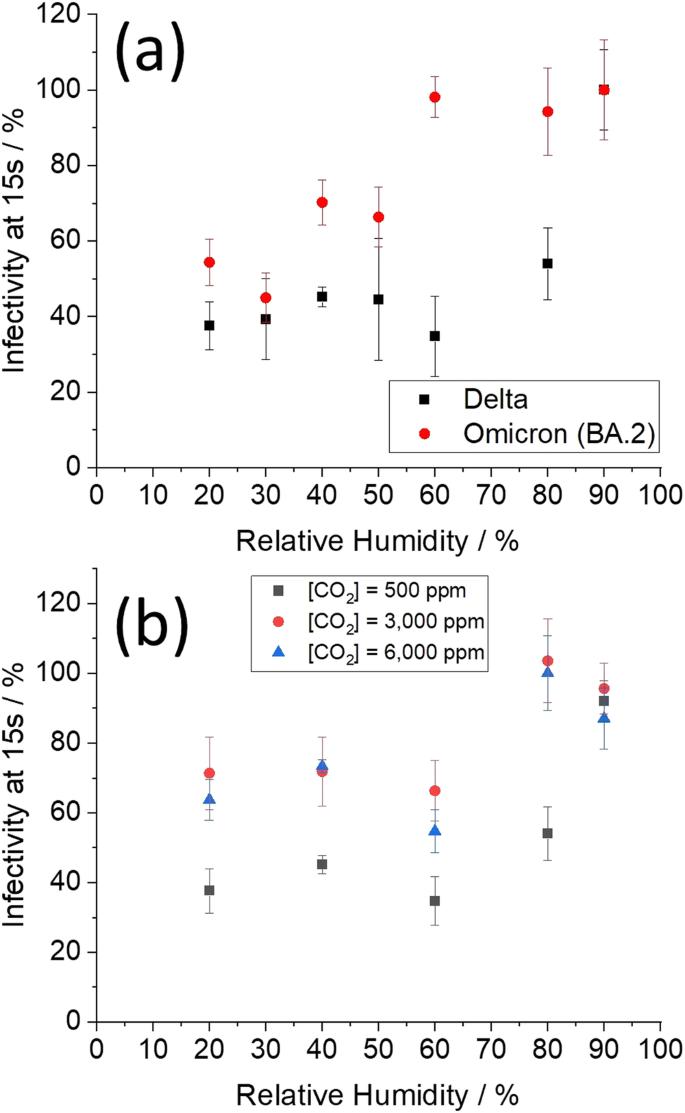
a Infectivity of the Delta and Omicron BA.2 variants at 15 s in ambient air as a function of relative humidity. b Infectivity of the Delta variant at 15 s as a function of relative humidity and [CO2(g)]. Values are means ± SE. A two-way ANOVA of the data in (b) indicated that both CO2(g) and RH are significant factors in predicting infectivity, but do not interact, meaning the effect of CO2(g) is similar at different RHs (all 80% and below). P-values: RH 0.016, CO2(g) n = 169 (independent samples). Source data are provided as a Source Data file.
Given that the BA.2 VOC is more robust than the Delta VOC over the 15 s time period, the impact of [CO2(g)] and [NaCl] on the aerostability of the Delta VOC have been explored further. The effect that a moderate increase in [CO2(g)] has on the aerostability of this SARS-CoV-2 VOC is reported in Fig. 3b. Regardless of RH, increasing the [CO2(g)] will drive the pH of an alkaline respiratory droplet towards neutral to some degree. As a result, at an RH of 80% and below, moderate increases in the [CO2(g)] are shown to increase viral aerostability. This increase in [CO2(g)] results in a doubling of the remaining aerosolized viral load after 15 s for all RH t-test (p = 5 × 10−4, with 27.5 effective degrees of freedom). At 90% RH, no viral decay was measured (Fig. 3a, b); droplets injected into an RH of 90% are still evaporating at 15 s and, thus, the conditions in the droplet are not sufficiently different to affect viral infectivity.
Risk of transmission is highly affected by ambient concentrations of CO2
The dependence of the overall risk of SARS-CoV-2 transmission on the explicit decay dynamics inferred from measurements with the CELEBS technique has been investigated using a Wells-Riley model38. Specifically, the impact of environmental factors such as [CO2(g)] and humidity on the likelihood of disease transmission have been explored. The Wells-Riley model is based on transmitted quanta that inherently assume a uniformity in a mixed room, only strictly true for small particles in the bronchiolar and laryngeal modes, both 50 µm diameter). We use the infectivity decay data from these large droplet measurements to inform estimates of transmission risk for the small aerosol fraction and estimate the relative changes in risk that result from changes in [CO2(g)]. It should be noted that the rate of pH reduction will be dependent on both droplet size and total acid content in the air, where smaller droplets will be neutralized at a faster rate. The effect of size was explored in this study to a limited degree and was found to have a minimal effect within the size range explored (Supplementary Fig. 6). The precise degree to which the droplet size affects the rate of neutralization (and subsequent increased viral aerostability) should be measured in the future.
Typically, models assume that the aerosolized viral decay has a half-life of 1.1 h39 when estimating the risk of COVID-19 transmission, a rate of decay which is negligible when compared to the effects of even the poorest ventilation. As shown in Fig. 1, viral decay dynamics are more complex than the assumed single exponential decay. To be clear, the rapid early decay in infectivity of aerosolized SARS-CoV-2 we report here, as well as previously14, does not contradict the consensus opinion that airborne transmission prevails as the dominant mode of transmission. Our objective here is to demonstrate that the decay dynamics reported in Fig. 1a, b are actually consistent with this consensus, especially in indoor environments. We therefore focus on the limit of a well-mixed indoor environment using the Wells-Riley framework and using our refined characterization of the infectivity decay rate.
Central to the Wells-Riley approach is the number of infectious units (“quanta”) that remain active in a room. In a ventilated environment, the probability that an aerosolized unit remains in the room after some time is described by Equation 2.
$${p}_{{active}}=1-\exp \left(\frac{-t}{{\tau }_{{vent}}}\right)$$
(2)
where \({\tau }_{{vent}}\) is the characteristic time to cycle air in the room. We consider typical ventilation rates for \({\tau }_{{vent}}^{-1}\) in the range 0.5–8 h−1 with smaller numbers indicating a poorly ventilated space40. Any effect of droplet removal by deposition which may occur for coarser droplets is ignored. The fraction of droplets remaining viable to initiate infection is \({p}_{{active}}I\), where I is their infectivity. Initially, we consider a fully recirculating ventilation system where the [CO2(g)] remains constant so that we can assume the decay in infectivity directly follows that reported from the CELEBS data; this set-up models a closed Heating, Ventilation and Air-Condition (HVAC) system. We assume the HVAC system perfectly filters the air of aerosol droplets, although this is a crude oversimplification41. Later we will allow for varying [CO2(g)] in order to model ventilation and mixing with an outdoor air source (from e.g., opening a window). For convenience, we fit the CELEBS data (Fig. 1a,b) with two exponentially decaying functions (details in Supplementary). In poorly ventilated environments, the viability of aerosolized virus is dominated by the intrinsic decay in infectivity (Fig. 4a). Air recirculation dominates in better-conditioned environments leading to convergence of the long-time decay (Fig. 4b). We compare these model predictions with a simple exponentially decaying infectivity with the widely assumed aerosolized half-life of 1.1 h31 from drum data.
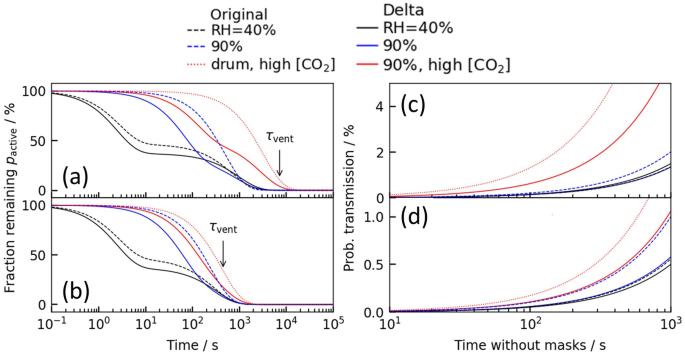
Fraction of infectious aerosol particles remaining in the a poorly ventilated (0.5 air changes per hour) and b well-ventilated (8 air changes per hour) classroom where “Time” is the time following exhalation of the infectious aerosol. Probability of onward transmission to a susceptible individual assuming a well-mixed c poorly ventilated (0.5 air changes per hour) and d well-ventilated (8 air changes per hour) environment, where “Time” is the time following occupation of the room. An infectious quanta production rate of 0.1s-1 is assumed (38). Decay profile in (a, b) is used to model the transmission risk in (c, d).
In the Wells-Riley model, the infection probability \({p}_{I}\) is assumed to depend exponentially on the number of infectious units (“quanta”) received \(n\), i.e. \({p}_{I}\left(t\right)=1-\exp \left(-n\left(t\right)\right)\). This exponential dose-response relationship is essentially a consequence of the independent action hypothesis42. The number of quanta n describes the number of infectious viral doses received, incorporating the effects of viral viability and ventilation. The typical number of quanta received increases linearly in time \(t\) as \(n\left(t\right)=c\dot{V}t\) where c is the concentration of quanta in the well-mixed air and \(\dot{V}\) is the minute volume of exhaled air (from breathing) which we take to be 7.5 L/min. The steady-state quanta concentration is found where \({p}_{{active}}I\) balances the rate that infectious quanta are produced and released into the environment (details in Supplementary). For illustration purposes, we consider a 10 × 10 × 3 = 300 m3 classroom with up to 40 occupants where there is a single infected individual. The quanta production rate by an infected individual is considered to be in the range 0.01–0.1 s−1 for SARS-CoV-239. We assume a value of 0.1 s−1 for illustrative purposes, with the understanding that this factor remains a major source of uncertainty in transmission models. The probability of onward transmission in a poorly ventilated classroom is similar for all datasets at low [CO2(g)] (Fig. 4c, blue and black lines) because only the long-time behavior matters in this well-mixed limit. By contrast, the probability of onward transmission rises much more rapidly for the high [CO2(g)] (Fig 4, red lines). The amplifying effect of [CO2(g)] is visible but less pronounced in a space with good ventilation using, e.g., an open window (Fig. 4d). The probability of transmission is sensitive to parameters with large uncertainties like the quanta production rate, so the absolute values of transmission probability in Fig. 4c, d should not be taken literally. Rather, the important point is that the relative difference in probability between low [CO2(g)] and high [CO2(g)] can be striking; even with good ventilation (Fig. 4d), the probability of onward transmission approximately doubles for [CO2(g)] = 3000 ppm over the [CO2(g)] = 500 ppm after ~15 min of exposure.
For risk management, we must consider the risk that any susceptible individual becomes infected, rather than just a single individual. The probability that at least one individual becomes infected is:
$$1-{\left(1-{p}_{I}\left(t\right)\right)}^{N-1}$$
(3)
where \(N\) is the room occupancy. As a measure of risk, we invert this relationship to determine the length of time the classroom space can be shared until there is a 50% chance that secondary transmission has occurred. The probability of a successful transmission (assuming a well-mixed environment) as a function of viral aerostability and ventilation is explored in Fig. 5. Estimates for the Delta variant at high [CO2(g)] are comparable in magnitude to predictions with a decay time assumed from the drum studies. By contrast, the effect of increased [CO2(g)] has a profound effect on the overall risk of transmission. Even in well-ventilated classrooms with 10 air changes per hour (in a recirculating system), we see that the time before an expected transmission occurs is approximately halved by raising the [CO2(g)].
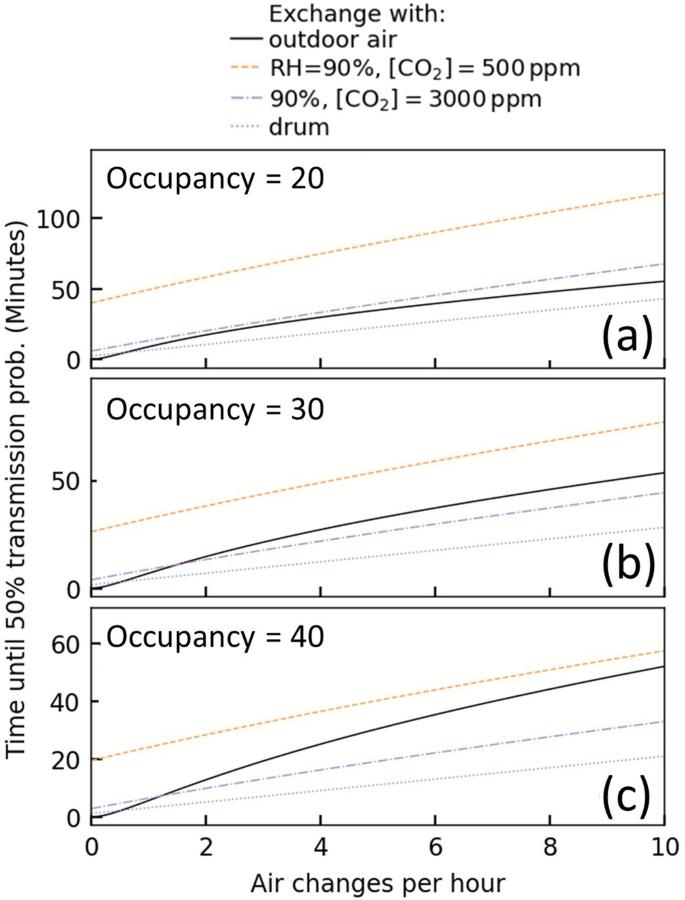
We combine estimates of the [CO2(g)] in the room with interpolations of the datasets for Delta variant’s infectivity at 90% RH to estimate the risk when exchanging with outdoor air (black, 40% RH, 450 ppm [CO2(g)]). This interpolation is purely intended to illustrate the nonlinear role of ventilation qualitatively, and so these numbers should not be taken literally. For comparison we also show the expectation at fixed low (purple) and high (orange) [CO2(g)] without any interpolation; this models recirculation flow within a HVAC system. The number of occupants is varied a 20, b 30, and c 40. An infectious quanta production rate of 0.1s-1 is assumed.
Sustained [CO2(g)] at a higher concentration at a fixed ventilation rate (e.g., more recirculation of room air and less mixing of fresh air) implies lower aerosol pH, greater survival, and shorter time until 50% transmission. Assuming a slower decay rate consistent with the drum data does not incorporate the rapid initial loss of infectivity which means, at the same quanta emission rate, there is more infectious virus and shorter time to reach 50% infectivity. Outdoor air lowers the CO2 concentration, ensuring the pH remains higher, leading to lower infectivity and longer time to reach same infectivity as ACH goes up.
Despite the rapid initial decay (Fig. 1a, b), the Wells-Riley model prediction estimates that long-distance transmission is possible. However, the Wells-Riley model neglects the short-time decay indicating that short-range airborne transmission route (from e.g., direct conversation) may be underestimated in these conventional approaches. Collectively, the risk estimations from the Wells-Riley model demonstrate the importance of ventilation in mitigating risk as it addresses aerosolized viral load on two fronts: firstly, the rate of loss of viral infectivity in the aerosol phase (e.g., lower [CO2(g)] increases decay rate) and, secondly, the physical reduction of the number of viral containing particles (e.g., displaced from the room). The Wells-Riley model demonstrates the importance of [CO2(g)] and RH on long-distance transmission risk. In the future, the effect the rapid loss of infectivity in the aerosol phase at low RH has on short-distance transmission risk should be explored using a CFD model.